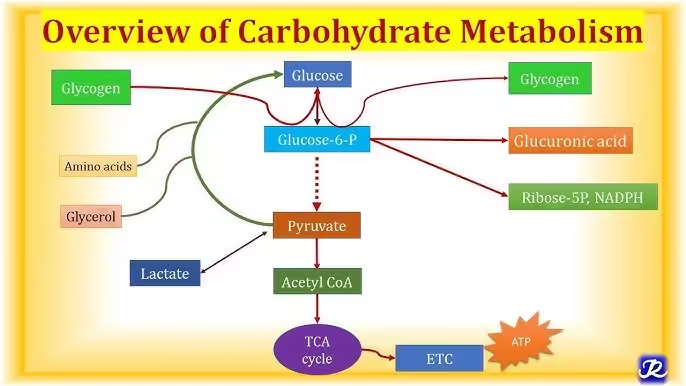
Digestion of Carbohydrates
The principal sites of carbohydrate digestion are the mouth and small intestine. The dietary carbohydrate consists of:
- Polysaccharides: Starch, glycogen, and cellulose
- Disaccharides: Sucrose, maltose, and lactose
- Monosaccharides: Mainly glucose and fructose.
-
Digestion in the Mouth
- Mechanical Breakdown: Chewing (mastication) physically breaks down food into smaller particles, increasing the surface area for enzymes to act on.
- Enzyme Activity: Salivary glands secrete salivary amylase (ptyalin), which hydrolyzes the glycosidic bonds in starch and glycogen, storing polysaccharides in plants and animals. This enzyme specifically breaks down α-1,4-glycosidic bonds.
- End Products in Mouth: Salivary amylase breaks starches down into maltose (a disaccharide of two glucose molecules), maltotriose (three glucose molecules), and α-limit dextrins (small branched fragments with α-1,6 branches). However, this breakdown is limited because food doesn’t stay in the mouth for long.
-
Digestion in the Stomach
- Acidic Environment: The stomach’s acidic environment (pH 1.5-3.5) inactivates salivary amylase. This acidity is necessary to unfold proteins for digestion but halts carbohydrate breakdown temporarily.
- Limited Carbohydrate Digestion: Unlike proteins and lipids, carbohydrates are not further digested in the stomach due to the lack of carbohydrate-specific enzymes in gastric juice.
-
Digestion in the Small Intestine
- Role of Pancreatic Amylase: Once the partially digested food (now called chyme) reaches the duodenum, the pancreas releases pancreatic amylase through the pancreatic duct. This enzyme is highly efficient at hydrolyzing α-1,4-glycosidic bonds, further breaking down any remaining polysaccharides into maltose, maltotriose, and α-limit dextrins.
- Brush Border Enzymes: The small intestine’s epithelial cells have microvilli forming the brush border, where final digestion occurs.
- Maltase: Hydrolyzes maltose into two glucose molecules.
- Sucrase: Splits sucrose (table sugar) into one glucose and one fructose molecule.
- Lactase: Breaks down lactose (milk sugar) into glucose and galactose. This enzyme is particularly important for infants who rely on milk as their primary nutrient source. Lactase deficiency leads to lactose intolerance.
- Isomaltase (or α-dextrinase): Hydrolyzes α-1,6-glycosidic bonds in α-limit dextrins, releasing individual glucose molecules.
-
Absorption in the Small Intestine
- Absorption of Glucose and Galactose: Glucose and galactose are absorbed through a sodium-glucose co-transporter protein called SGLT1. This process involves secondary active transport, which requires an electrochemical gradient of sodium (Na⁺) across the enterocyte membrane. Sodium is pumped out of the cell by the Na⁺/K⁺ ATPase pump, which creates a gradient that drives glucose and galactose into the cell alongside Na⁺.
- Absorption of Fructose: Fructose is absorbed via facilitated diffusion through a transporter protein called GLUT5. This type of transport does not require energy but relies on the concentration gradient of fructose.
- Exit from Enterocytes to Bloodstream: Once inside the enterocytes (intestinal cells), glucose, galactose, and fructose exit the cells into the bloodstream through another transporter protein, GLUT2, located on the basolateral membrane of the enterocyte.
-
Transport to the Liver
- Portal Vein Transport: The absorbed monosaccharides (glucose, galactose, and fructose) travel via the hepatic portal vein to the liver. In the liver:
- Galactose and Fructose Conversion: Both galactose and fructose are converted into glucose derivatives via enzymatic pathways in hepatocytes (liver cells).
- Glucose: Glucose can be stored as glycogen in the liver, used directly for energy, or released into the bloodstream to maintain blood glucose levels, essential for energy supply to other organs, especially the brain.
-
Systemic Distribution and Cellular Uptake
- Circulating Blood Glucose: Glucose enters the general circulation after processing the liver. Tissues and organs can take up glucose based on their energy needs.
- Hormonal Regulation: Insulin and glucagon regulate blood glucose levels. Insulin is released by the pancreas in response to high blood glucose, facilitating glucose uptake by cells, especially muscle and adipose tissue, via GLUT4 transporters.
- Storage and Energy Use: Glucose can be used for immediate energy through glycolysis, stored as glycogen in muscles and liver, or converted to fat when in excess.
Glycolysis:
-
Definition of Glycolysis
- Glycolysis is a metabolic pathway that breaks down one glucose molecule (a 6-carbon sugar) into two pyruvate molecules (a 3-carbon compound), generating energy from ATP and NADH. It is the first step in cellular respiration and provides energy for cells in oxygen’s presence (aerobic conditions) and absence (anaerobic conditions).
- Importance: Glycolysis is crucial for energy production, particularly for cells lacking mitochondria (e.g., red blood cells) or during anaerobic conditions (e.g., intense muscle activity).
-
Location of Glycolysis
- Cellular Location: Glycolysis occurs in the cytoplasm of all cells.
- Tissue Location: It is active in nearly all tissues, with especially high activity in tissues that rely heavily on glucose for energy, such as muscle tissue, the brain, and red blood cells.
-
Reaction Steps of Glycolysis
Glycolysis consists of ten enzyme-catalyzed reactions, split into two main phases: an energy investment phase and an energy payoff phase.
Step-by-Step Breakdown of Glycolysis:
- Energy Investment Phase:
- Step 1: Glucose is phosphorylated by hexokinase (or glucokinase in the liver), using ATP to form glucose-6-phosphate.
- Step 2: Glucose-6-phosphate is converted into fructose-6-phosphate by the enzyme phosphoglucose isomerase.
- Step 3: Fructose-6-phosphate is phosphorylated by phosphofructokinase-1 (PFK-1), using another ATP to form fructose-1,6-bisphosphate. This step is a major regulatory point.
- Step 4: Fructose-1,6-bisphosphate is split into two 3-carbon molecules, glyceraldehyde-3-phosphate (G3P) and dihydroxyacetone phosphate (DHAP), by aldolase.
- Step 5: DHAP is converted into G3P by triose phosphate isomerase, resulting in two G3P molecules that enter the next phase.
- Energy Payoff Phase:
- Step 6: Each G3P is oxidized and phosphorylated by glyceraldehyde-3-phosphate dehydrogenase, producing 1,3-bisphosphoglycerate and NADH from NAD⁺.
- Step 7: 1,3-bisphosphoglycerate donates a phosphate to ADP (via phosphoglycerate kinase), forming ATP and 3-phosphoglycerate. This is the first ATP-generating step.
- Step 8: 3-phosphoglycerate is converted to 2-phosphoglycerate by phosphoglycerate mutase.
- Step 9: 2-phosphoglycerate is dehydrated by enolase, forming phosphoenolpyruvate (PEP).
- Step 10: PEP donates a phosphate to ADP (via pyruvate kinase), forming another ATP and pyruvate. This is the second ATP-generating step.
-
Regulation of Glycolysis
Glycolysis is regulated at key enzymatic steps to meet the cell’s energy needs. The main regulatory mechanisms include:
- Allosteric Regulation:
- Hexokinase is inhibited by its product, glucose-6-phosphate, which prevents excessive glucose uptake when downstream glycolytic intermediates accumulate.
- Phosphofructokinase-1 (PFK-1): The primary control point of glycolysis. PFK-1 is:
- Activated by AMP and fructose-2,6-bisphosphate (indicating low energy or high need for glucose metabolism).
- Inhibited by ATP and citrate (indicating sufficient energy supply or abundance of biosynthetic precursors).
- Pyruvate kinase: Inhibited by ATP and alanine (signals of high energy status and building blocks), and activated by fructose-1,6-bisphosphate (a feedforward activator ensuring that downstream steps keep pace with PFK-1 activity).
- Hormonal Regulation:
- Insulin: Promotes glycolysis by increasing glucose uptake and activating glycolytic enzymes, particularly in muscle and adipose tissue.
- Glucagon: Inhibits glycolysis in the liver by reducing the levels of fructose-2,6-bisphosphate, which decreases PFK-1 activity.
TCA Cycle:
-
Definition of the TCA Cycle
- The Tricarboxylic Acid (TCA) Cycle, also known as the Krebs Cycle or Citric Acid Cycle, is a central metabolic pathway that oxidizes acetyl-CoA (derived from carbohydrates, fats, and proteins) into carbon dioxide while generating high-energy molecules NADH FADH₂, and GTP (or ATP). These products drive ATP synthesis in oxidative phosphorylation.
- Importance: The TCA cycle is crucial for cellular respiration, energy production, and providing intermediates for biosynthetic pathways.
-
Location of the TCA Cycle
- Cellular Location: The TCA cycle occurs in the mitochondrial matrix of eukaryotic cells.
- Tissue Location: It is active in most tissues, with high activity in energy-demanding tissues such as the heart, liver, kidneys, and muscles.
-
Reactions of the TCA Cycle
The TCA cycle involves eight enzyme-catalyzed reactions that regenerate oxaloacetate, which can then combine with a new acetyl-CoA molecule to continue the cycle.
Step-by-Step Breakdown of the TCA Cycle:
- Condensation of Acetyl-CoA and Oxaloacetate:
- Enzyme: Citrate synthase
- Reaction: Acetyl-CoA (2 carbons) combines with oxaloacetate (4 carbons) to form citrate (6 carbons), releasing CoA.
- Isomerization of Citrate to Isocitrate:
- Enzyme: Aconitase
- Reaction: Citrate is converted to isocitrate through a two-step dehydration-rehydration reaction.
- Oxidative Decarboxylation of Isocitrate:
- Enzyme: Isocitrate dehydrogenase
- Reaction: Isocitrate is oxidized to α-ketoglutarate (5 carbons), producing NADH and releasing CO₂.
- Oxidative Decarboxylation of α-Ketoglutarate:
- Enzyme: α-Ketoglutarate dehydrogenase
- Reaction: α-Ketoglutarate is converted to succinyl-CoA (4 carbons), producing NADH and releasing a second CO₂ molecule.
- Conversion of Succinyl-CoA to Succinate:
- Enzyme: Succinyl-CoA synthetase
- Reaction: Succinyl-CoA is converted to succinate, generating GTP (or ATP) and releasing CoA.
- Oxidation of Succinate to Fumarate:
- Enzyme: Succinate dehydrogenase
- Reaction: Succinate is oxidized to fumarate, producing FADH₂.
- Hydration of Fumarate to Malate:
- Enzyme: Fumarase
- Reaction: Fumarate is hydrated to form malate.
- Oxidation of Malate to Oxaloacetate:
- Enzyme: Malate dehydrogenase
- Reaction: Malate is oxidized to regenerate oxaloacetate, producing the third NADH.
-
Regulation of the TCA Cycle
The TCA cycle is tightly regulated to match energy demand and ensure balance in metabolic intermediates.
Regulation by Enzyme Activity:
- The TCA cycle is primarily regulated at three irreversible steps catalyzed by citrate synthase, isocitrate dehydrogenase, and α-ketoglutarate dehydrogenase. These enzymes respond to allosteric effectors and energy signals.
- Citrate Synthase:
- Inhibited by ATP, NADH, and citrate (indicating high energy or sufficient cycle intermediates).
- Activated by ADP (indicating low energy availability).
- Isocitrate Dehydrogenase:
- Activated by ADP and Ca²⁺ (particularly in muscle cells).
- Inhibited by ATP and NADH.
- α-Ketoglutarate Dehydrogenase:
- Activated by Ca²⁺ (important during muscle contraction, which increases energy demands).
- Inhibited by NADH and succinyl-CoA (indicating high cycle intermediates and sufficient energy).
Product Inhibition and Feedback Regulation:
- NADH: High levels of NADH (from energy abundance) inhibit several enzymes in the TCA cycle, including citrate synthase, isocitrate dehydrogenase, and α-ketoglutarate dehydrogenase.
- ATP: Acts as an allosteric inhibitor of enzymes like citrate synthase and isocitrate dehydrogenase.
- Intermediates: Some intermediates like succinyl-CoA can feedback-inhibit earlier enzymes (such as α-ketoglutarate dehydrogenase) to prevent excessive accumulation.
Substrate Availability:
- Oxaloacetate and Acetyl-CoA Availability: The entry of acetyl-CoA into the cycle depends on the availability of oxaloacetate, which links glycolysis and gluconeogenesis to the TCA cycle. The TCA cycle slows down if oxaloacetate levels are low (e.g., in fasting states).
Hormonal and Cellular Energy Demand Regulation:
- Although the TCA cycle is not directly regulated by hormones, cellular conditions like high ATP demand (e.g., during exercise) increase glycolysis and acetyl-CoA production, thus enhancing TCA cycle activity.
- Calcium ions (Ca²⁺): During muscle contraction, increased Ca²⁺ levels activate key enzymes (like isocitrate dehydrogenase and α-ketoglutarate dehydrogenase), ramping up the cycle’s activity to meet the energy needs.
HMP Pathway:
-
Definition of the HMP Pathway
- The Hexose Monophosphate (HMP) Pathway, also known as the Pentose Phosphate Pathway (PPP) or Phosphogluconate Pathway, is an alternative metabolic pathway for glucose oxidation. Instead of generating ATP, the HMP pathway produces NADPH (essential for biosynthetic reactions and antioxidant defense) and ribose-5-phosphate (a precursor for nucleotide synthesis).
- Importance: The HMP pathway is crucial for producing reducing power (in the form of NADPH) for anabolic processes (such as fatty acid and steroid synthesis) and maintaining cellular redox balance, as well as for synthesizing ribose-5-phosphate for DNA and RNA production.
-
Location of the HMP Pathway
- Cellular Location: The HMP pathway occurs in the cytoplasm of cells.
- Tissue Location: It is especially active in tissues that require high levels of NADPH or nucleotide synthesis, such as:
- Liver (for fatty acid and cholesterol synthesis),
- Adipose tissue (for fatty acid synthesis),
- Adrenal glands (for steroid synthesis),
- Red blood cells (for antioxidant defense), and
- Rapidly dividing cells (such as those in bone marrow or tumors, which need nucleotides for DNA replication).
-
Reactions of the HMP Pathway
The HMP pathway is divided into two phases: the oxidative phase, which generates NADPH, and the non-oxidative phase, which produces ribose-5-phosphate and other sugars.
Step-by-Step Breakdown of the HMP Pathway:
- Oxidative Phase:
- Step 1: Glucose-6-phosphate is oxidized by glucose-6-phosphate dehydrogenase (G6PD), reducing NADP⁺ to NADPH and forming 6-phosphogluconolactone.
- Step 2: 6-Phosphogluconolactone is hydrolyzed by 6-phosphogluconolactonase to form 6-phosphogluconate.
- Step 3: 6-Phosphogluconate is further oxidized and decarboxylated by 6-phosphogluconate dehydrogenase, generating another NADPH, forming ribulose-5-phosphate, and releasing CO₂.
- Net Gain of the Oxidative Phase: For each molecule of glucose-6-phosphate, two molecules of NADPH and one molecule of CO₂ are produced.
- Non-Oxidative Phase:
- Ribulose-5-phosphate (from the oxidative phase) is converted into ribose-5-phosphate by phosphopentose isomerase or xylulose-5-phosphate by phosphopentose epimerase.
- Ribose-5-phosphate can be used for nucleotide synthesis, or it can be further metabolized.
- In tissues that do not require ribose-5-phosphate, the non-oxidative phase interconverts sugar phosphates (using transketolase and transaldolase enzymes) to produce intermediates that can enter glycolysis or gluconeogenesis:
- Transketolase transfers 2-carbon units.
- Transaldolase transfers 3-carbon units.
- The products include fructose-6-phosphate and glyceraldehyde-3-phosphate, which can re-enter glycolysis.
-
Regulation of the HMP Pathway
The HMP pathway is regulated mainly through the availability of its substrate, glucose-6-phosphate, and the cell’s demand for NADPH and ribose-5-phosphate. Key regulatory mechanisms include:
Allosteric Regulation:
- The key regulatory enzyme in the HMP pathway is glucose-6-phosphate dehydrogenase (G6PD), which catalyzes the first step in the oxidative phase and is:
- Activated by NADP⁺: A high ratio of NADP⁺/NADPH activates G6PD, increasing NADPH production when the cell requires reduced power.
- Inhibited by NADPH: High levels of NADPH inhibit G6PD, reducing NADPH production when it is abundant.
Feedback Mechanisms Based on Cellular Needs:
- NADPH Demand: If the cell has a high demand for NADPH (e.g., for fatty acid synthesis or antioxidant defense), the oxidative phase is more active in producing NADPH.
- Ribose-5-Phosphate Demand: If the cell requires more ribose-5-phosphate (e.g., for nucleotide synthesis in rapidly dividing cells), it can increase the flow through the non-oxidative phase, bypassing the oxidative phase if NADPH levels are sufficient.
Hormonal Regulation:
- Insulin stimulates glucose-6-phosphate dehydrogenase expression, increasing the flow through the HMP pathway in tissues involved in biosynthesis (such as liver and adipose tissue). This regulation aligns the path with the cell’s anabolic and energy-storage functions following carbohydrate intake.
Diabetes Mellitus:
- Overview
- Diabetes Mellitus (DM) is a chronic metabolic disorder characterized by high blood glucose (sugar) levels due to insulin deficiency, insulin resistance, or both.
- Insulin, a hormone the pancreas produces, regulates blood glucose levels by facilitating cellular glucose uptake for energy or storage.
- Uncontrolled diabetes can lead to severe complications affecting various organs, including the heart, kidneys, eyes, and nerves.
-
Types of Diabetes Mellitus
There are several types of diabetes mellitus, the most common of which include:
- Type 1 Diabetes (T1D): An autoimmune condition where the immune system attacks and destroys insulin-producing beta cells in the pancreas. This type requires lifelong insulin therapy and typically appears in childhood or early adulthood.
- Type 2 Diabetes (T2D): The most common form of diabetes, characterized by insulin resistance (cells respond poorly to insulin) and eventual insulin deficiency. T2D is often associated with obesity, physical inactivity, and genetic predisposition, and it usually develops in adulthood, though it is increasingly diagnosed in children.
- Gestational Diabetes (GDM): This type develops during pregnancy and usually resolves after childbirth, though it increases the risk of developing T2D later in life.
- Other Specific Types: These include monogenic diabetes, secondary diabetes due to other medical conditions (like Cushing’s syndrome or cystic fibrosis), and diabetes caused by certain medications or pancreatic diseases.
-
Causes and Risk Factors
- Type 1 Diabetes: Caused by autoimmune destruction of pancreatic beta cells. Risk factors include genetic predisposition, family history, and possible environmental triggers like viral infections.
- Type 2 Diabetes: Caused by a combination of genetic factors and lifestyle influences. Major risk factors include:
- Obesity: Excess fat, particularly around the abdomen, increases insulin resistance.
- Physical Inactivity: Increases risk by contributing to obesity and reducing insulin sensitivity.
- Genetics and Family History: Certain genes increase susceptibility.
- Age: Risk increases with age, especially after 45.
- Other Factors: Poor diet, hypertension, high cholesterol, and certain ethnic backgrounds (such as African, Hispanic, and Asian ancestry).
- Gestational Diabetes: Hormonal changes during pregnancy can impair insulin action, leading to gestational diabetes. Risk factors include obesity, advanced maternal age, and a family history of diabetes.
-
Symptoms
Common symptoms of diabetes mellitus include:
- Frequent urination (polyuria)
- Excessive thirst (polydipsia)
- Extreme hunger (polyphagia)
- Unexplained weight loss
- Fatigue
- Blurred vision
- Slow-healing sores or frequent infections
- Numbness or tingling in hands and feet (mainly in T2D)
In Type 1 Diabetes, symptoms typically develop suddenly; in Type 2 Diabetes, symptoms may appear gradually over time.
-
Diagnosis
Diagnosis of diabetes mellitus is based on several blood tests, including:
- Fasting Plasma Glucose (FPG): Measures blood glucose after an 8-hour fast. A level of 126 mg/dL or higher on two separate occasions indicates diabetes.
- Oral Glucose Tolerance Test (OGTT): Measures blood glucose two hours after consuming a glucose-rich drink. A level of 200 mg/dL or higher indicates diabetes.
- A1C Test (Glycated Hemoglobin): Reflects average blood glucose over the past 2-3 months. An A1C level of 6.5% or higher indicates diabetes.
- Random Plasma Glucose Test: A level of 200 mg/dL or higher and symptoms may indicate diabetes.
-
Complications
- Acute Complications Include diabetic ketoacidosis (DKA) and hyperosmolar hyperglycemic state (HHS), which can be life-threatening if untreated.
- Chronic Complications: These arise from prolonged high blood glucose levels and may include:
- Cardiovascular Disease: Increased risk of heart disease and stroke.
- Neuropathy: Nerve damage, often in the legs and feet, leading to numbness or pain.
- Nephropathy: Kidney damage, which can lead to kidney failure.
- Retinopathy: Eye damage leading to blindness.
- Foot Damage: Poor blood flow and nerve damage can result in severe foot infections, often requiring amputation.
-
Treatment and Management
Treatment and management of diabetes depend on the type, severity, and individual patient needs. Key components of diabetes care include:
- Lifestyle Modifications:
- Diet: A balanced diet with controlled carbohydrates, low refined sugars, high fiber, and moderate protein and fat intake is essential.
- Exercise: Regular physical activity improves insulin sensitivity and helps control blood glucose levels.
- Medications:
- Type 1 Diabetes: Insulin therapy is essential and is administered through injections or an insulin pump.
- Type 2 Diabetes: Often managed with oral medications such as metformin, sulfonylureas, or GLP-1 agonists, and sometimes requires insulin.
- Blood Glucose Monitoring: Regular monitoring helps patients keep track of glucose levels and adjust their lifestyle or medication accordingly.
- Weight Management: Weight loss is beneficial, particularly in T2D, where it can sometimes improve insulin sensitivity and even lead to remission.
- Education and Support: Diabetes education, along with support from healthcare providers, family, and support groups, helps individuals manage their condition and adhere to treatment regimens.
-
Prevention
While Type 1 diabetes cannot be prevented, Type 2 diabetes risk can be reduced by maintaining a healthy weight, eating a balanced diet, staying active, and monitoring blood glucose levels regularly if at high risk.
Blood glucose regulation
Blood glucose regulation is a complex physiological process that maintains blood glucose levels within a narrow range. This is essential for energy homeostasis and involves multiple hormones, organs, and signaling pathways that coordinate the body’s response to rising or falling blood glucose levels.
-
Blood Glucose Levels and Normal Range
- Normal Fasting Blood Glucose: Around 70-100 mg/dL.
- Postprandial (after eating) Blood Glucose: Typically does not exceed 140 mg/dL in healthy individuals.
- Homeostasis Importance: The brain and nervous system rely on glucose as a primary energy source, making precise glucose regulation critical.
-
Key Hormones in Blood Glucose Regulation
The two primary hormones involved in blood glucose regulation are insulin and glucagon, both produced by the pancreas. Additional hormones such as epinephrine, cortisol, and growth hormone also play supportive roles.
- Insulin: A hormone produced by beta cells in the pancreas, insulin is the primary regulator that lowers blood glucose.
- Glucagon: A hormone produced by alpha cells in the pancreas, glucagon raises blood glucose by promoting the release of stored glucose from the liver.
- Epinephrine (adrenaline): Released by the adrenal glands during stress, it increases blood glucose by promoting glycogen breakdown in the liver.
- Cortisol: A glucocorticoid hormone that increases blood glucose by stimulating gluconeogenesis in the liver, particularly in response to long-term stress.
- Growth Hormone: Produced by the pituitary gland, growth hormone raises blood glucose by reducing glucose uptake in tissues and promoting lipolysis.
-
Mechanisms of Blood Glucose Regulation
-
After Eating (Postprandial State)
- Increased Blood Glucose: After a meal, carbohydrates are broken down into glucose, increasing blood glucose levels.
- Insulin Secretion:
- Rising blood glucose stimulates the pancreatic beta cells to release insulin.
- Insulin binds to insulin receptors on cell surfaces, primarily in muscle, adipose, and liver tissues.
- Insulin’s Actions:
- Glucose Uptake: Insulin promotes glucose uptake into cells, especially in muscle and adipose tissue, by increasing the number of glucose transporter proteins (GLUT-4) on cell membranes.
- Glycogenesis: Insulin stimulates the liver to convert glucose into glycogen (glycogenesis) for storage.
- Inhibition of Gluconeogenesis and Glycogenolysis: Insulin suppresses glucose production in the liver by inhibiting gluconeogenesis (glucose synthesis from non-carbohydrate sources) and glycogenolysis (breakdown of glycogen to glucose).
- Lipogenesis: In adipose tissue, insulin promotes the storage of excess glucose as fat (lipogenesis).
- Result: The combined actions of insulin lower blood glucose levels back to a normal range.
During Fasting or Between Meals (Post-absorptive State)
- Decreased Blood Glucose: The pancreas reduces insulin and glucagon secretion when blood glucose levels drop (e.g., several hours after a meal).
- Glucagon Secretion:
- Low blood glucose stimulates alpha cells in the pancreas to release glucagon.
- Glucagon’s Actions:
- Glycogenolysis: In the liver, glucagon promotes the breakdown of glycogen into glucose, which is released into the bloodstream to raise blood glucose.
- Gluconeogenesis: Glucagon stimulates gluconeogenesis, which produces glucose from amino acids, lactate, and other substrates in the liver.
- Result: These processes increase blood glucose to maintain adequate levels of energy.
In Response to Stress or Exercise
- Epinephrine:
- During physical activity or stress, the adrenal glands release epinephrine.
- Epinephrine stimulates glycogen breakdown in the liver and muscle, quickly increasing blood glucose to meet the higher energy demands.
- Epinephrine also suppresses insulin secretion, ensuring glucose is readily available for muscle cells rather than being stored.
- Cortisol:
- Released by the adrenal cortex in response to long-term stress, cortisol promotes gluconeogenesis in the liver and reduces glucose uptake by tissues.
- This results in elevated blood glucose levels during prolonged stress.
- Growth Hormone:
- In periods of stress or fasting, growth hormone reduces glucose uptake in muscle and adipose tissue. It stimulates lipolysis, thereby increasing the availability of fatty acids as an alternative energy source.
- Regulation Pathways of Insulin and Glucagon Secretion
- Negative Feedback Mechanism: Blood glucose levels influence insulin and glucagon release through a feedback loop.
- High Blood Glucose: Stimulates insulin release and inhibits glucagon.
- Low Blood Glucose: Inhibits insulin release and promotes glucagon release.
- Incretins: Gut hormones such as GLP-1 (glucagon-like peptide-1) are released in response to food intake and enhance insulin secretion from the pancreas, regulating postprandial glucose levels.
- Dysregulation of Blood Glucose: Diabetes Mellitus
- Type 1 Diabetes (T1D): Autoimmune destruction of beta cells leads to insulin deficiency. Blood glucose regulation is impaired, causing chronic hyperglycemia.
- Type 2 Diabetes (T2D): Insulin resistance and eventual beta-cell dysfunction result in impaired glucose uptake and chronic hyperglycemia.
- Complications of Dysregulation: Prolonged hyperglycemia damages blood vessels, nerves, and various organs, leading to complications such as cardiovascular disease, neuropathy, nephropathy, and retinopathy.